Investigation of All-Optical Resonances for Compact Atomic Clocks
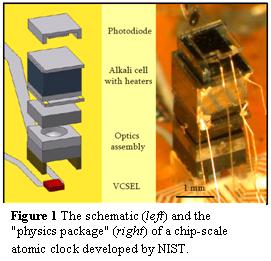
There is great current interest in developing compact, robust atomic clocks with low power consumption
and fractional frequency stability better than 10-12
(equivalent to accumulated error less than 1 second in 1000 years) for a wide variety of applications,
such as global positioning systems or telecommunication. Precise time keeping is based on the accurate
measurement of a resonance transition frequency between two ground-state hyperfine levels of certain
atomic species (hydrogen or an alkali-metal atom). This resonance, which is in the microwave range (1-10GHz),
is traditionally detected by means of the classical magnetic resonance technique [1].
In such a case, however, the size of the clock cannot be smaller than several centimeters
(for alkali-metals Rubidium or Cesium) because of the voluminous microwave cavity.
In the last decade there has been great progress towards the development of atomic clocks that are
based on all-optical probing of microwave atomic transitions in sub-mm size vapor cells [2,3].
A sample of such prototype, developed in National Institute of Standards and Technology (NIST), is shown in Fig.1. (
Click here to read more about NIST chip-scale atomic clock project.) Simultaneous interaction of an alkali-metal atoms with two
laser fields allows manipulation of the atomic quantum state if frequencies of optical fields matches
the resonance frequency between two hyperfine states of the ground electron state.
Under these resonance conditions optical properties of atoms become very sensitive to phase and/or frequency
differences between two optical fields, producing narrow resonances in the transmitted laser intensity spectrum.
The position of this resonance is determined by the hyperfine atomic transition, and thus the frequency
difference between two optical fields can be locked to the corresponding atomic transition for stable
microwave frequency reference, as shown in Fig.2. All-optical interaction schemes do not require
the bulky microwave cavity used in traditional clock designs and allow further miniaturization up to a
sub-cubic centimeter device volume.
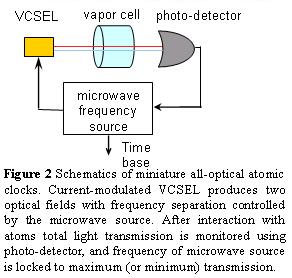
Existing compact all-optical atomic clocks rely on a vertical cavity surface emitting laser (VCSEL) to
produce two or more optical fields with well-controlled frequency differences in the microwave regime [5,6].
VCSEL is a type of semiconductor laser diode with laser beam emission perpendicular to the top surface, contrary
to the conventional diode lasers which emit from surfaces formed by cleaving the individual chip out of a wafer.
Apart from low power consumption and good single-mode (i.e. single-frequency) operation, VCSELs allow efficient
phase modulation of the output radiation at microwave frequencies up to several GHz through direct current modulation.
Such phase modulation in the frequency domain is equivalent to a comb of electromagnetic fields separated by a
modulation frequency, and thus two or more comb components can be used to produce two-photon optical resonances.
The recently demonstrated compact atomic clock prototypes are based on coherent population trapping
[7]. They have reached their expected stability limit of approximately 4⋅10-11Hz-1/2 [2,3,4].
The goal of my research is to invetigate and evaluate various all-optical two- and
three-photon resonances (such as three-photon absorption N-resonance) which will allow further improvements in stability of atomic clocks. I will use current-modulated VCSEL radiation to: a) study various all-optical two-photon transitions in Rb vapor; b) optimize the resonance parameter for better clock performance; c) lock the laser system to the atomic resonance in question and measure stability of such system.
References
[1] J. Vanier, C. Audoin, The Quantum Physics of Atomic Frequency Standards, (Adam Hilger, Bristol, UK, 1989)
[2] S. Knappe, V. Gerginov, P. D. D. Schwindt, V. Shah, H. G. Robinson, L. Hollberg, and J. Kitching, Opt. Lett. 30, 2351 (2005).
[3] R. Lutwak, D. Emmons, T. English, W. Riley, A. Duwel, M. Varghese, D.K. Serkland, G.M. Peake, in Proceedings of the 35th Precise Time and Time Interval (PTTI) Systems and Applications Meeting, 1 (2004)
[4] J. Vanier, M. Levine, S. Kendig, D. Janssen, C. Everson, and M. Delaney, in Proceedings of the IEEE International Ultrasonics, Ferroelectric and Frequency
Control Symposium, 1 (2004).
[5] C. Affolderbach, A. Nagel, S. Knappe, C. Jung, D. Wiedenmann, R. Wynands,
Appl. Phys. B 70, 407 (2000).
[6] J. Kitching, S. Knappe, N. Vukicevic, L. Hollberg, R. Wynands, W. Weidemann
IEEE Trans. Instrum. Meas. 49, 1313 (2000).
[7] J. Vanier, Appl. Phys. B 81, 421 (2005).
|